Exploring the Depths of Spectroscopy Instrumentation
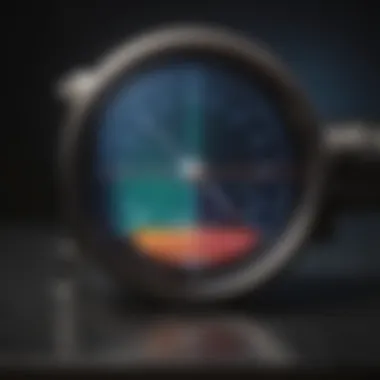
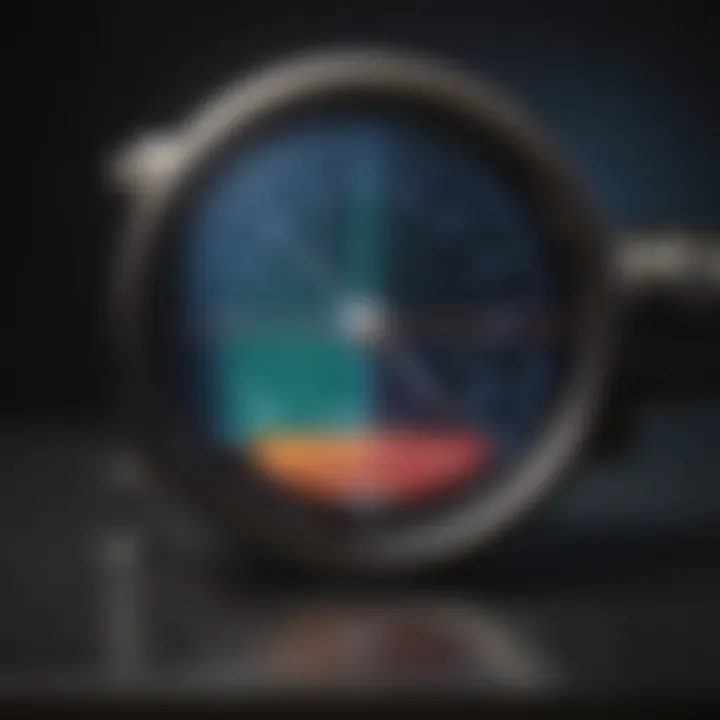
Intro
Spectroscopy instrumentation is a vital area in multiple scientific fields, providing insights into the composition and properties of materials. The principles governing spectroscopy allow researchers to analyze substances at a molecular or atomic level, leading to applications in chemistry, biology, material science, and environmental science. Understanding the various techniques and instruments can greatly enhance the ability to tackle complex research problems.
Research Context
Background Information
Spectroscopy has its roots in the study of light and matter interactions. Basic principles include absorption, emission, and scattering of light. Different spectroscopic techniques use these phenomena to provide details about various samples. Each method varies in its operating principles, instrumentation, and application areas.
Techniques such as infrared (IR) spectroscopy, nuclear magnetic resonance (NMR), and mass spectrometry, each have unique mechanisms of operation. These methods not only help in qualitative analysis but also in quantitative assessments, contributing to fields like drug discovery, environmental monitoring, and quality control in manufacturing.
Importance of the Study
The significance of spectroscopy in todayβs research landscape cannot be overstated. It serves as a cornerstone for both academic research and industrial applications. Understanding spectroscopy instrumentation can lead to advancements in methods that facilitate improved data collection. Furthermore, as technology advances, new tools and approaches emerge to refine spectral analysis.
An in-depth understanding of these advancements opens avenues for innovation. The integration of spectroscopy with automation and software tools enhances research capability, making studies faster and more accurate. This creates an environment conducive to exploring new scientific frontiers.
"Spectroscopy not only reveals the inner workings of materials but also shapes the methodologies and strategies in research."
Discussion
Interpretation of Results
Incorporating various spectroscopy techniques allows researchers to obtain complementary data, thus enriching the interpretation of results. Analyzing certain compounds with NMR can provide structural information that aids in the comprehension of their behavior under different conditions. Similarly, using mass spectrometry to identify molecular weights alongside IR spectroscopy for functional group identification can provide a complete chemical profile of a sample.
Comparison with Previous Research
Historically, spectroscopy was limited by available technologies and methodologies. Early instruments were often bulky and less sensitive. Recent innovations in detection methods and equipment miniaturization have vastly improved data quality and accessibility. Current studies show a significant increase in the accuracy of spectroscopic measurements when compared to past data.
The integration of machine learning with spectroscopy is an emerging area, highlighting the evolution of data interpretation. This allows for the handling of large datasets, providing insights that were previously unattainable. Each of these advancements ensures that spectroscopy remains relevant and critical in the landscape of scientific research.
Intro to Spectroscopy
The study of spectroscopy is fundamental to understanding the interaction between light and matter. Its importance cannot be overstated, as it serves as a bridge to deciphering complex molecular characteristics. Through this introduction, one gains insights into the underlying principles that govern diverse spectroscopic techniques, spanning from chemical analysis to medical applications. This foundation lays the groundwork for the comprehensive exploration of instrumentation, methods, and their implications in varied scientific endeavors.
Definition and Significance
Spectroscopy can be defined as the study of the interaction of electromagnetic radiation with matter. Specifically, it investigates how various wavelengths of light affect atoms and molecules, leading to transitions between energy levels. The significance of spectroscopy is vast. It allows scientists to identify and quantify substances, understand chemical reactions, and analyze physical properties. For researchers and professionals, the ability to derive qualitative and quantitative data from spectroscopic methods is invaluable.
The use of spectroscopy spans numerous fields, including chemistry, physics, biology, and environmental science. It enables the assessment of sample composition, the examination of structural properties, and the monitoring of changes over time. Spectroscopy is pivotal not only in research and development but also in quality control in industries such as pharmaceuticals, food, and materials science.
Historical Development
The historical development of spectroscopy is a narrative that illustrates both scientific curiosity and technological innovation. Beginning with Isaac Newton's work in the late 17th century, the field saw a shift as scientists began to systematically investigate light. Newton's prism experiments demonstrated the dispersion of light into a spectrum, setting the foundation for subsequent discoveries.
Advancements continued throughout the 19th century, with notable contributions from figures like Joseph von Fraunhofer, who characterized spectral lines in sunlight. This was a significant leap in recognizing that different elements emit distinct spectra. Later, the establishment of atomic theory further underpinned the connection between spectral data and elemental composition.
The 20th century brought about significant technological advancements. The development of laser technology revolutionized spectroscopy, enhancing precision and allowing for new applications in various fields, from chemical analysis to biomedical studies. Modern spectroscopy continues to evolve, integrating complex instrumentation and software for enhanced sensitivity and resolution.
"The evolution of spectroscopy is not merely a history of instruments and techniques. It embodies the interplay of scientific discovery and the relentless quest for knowledge across disciplines."
In summary, spectroscopy is a critical field of study that has developed over centuries, shaping our understanding of matter at a fundamental level. Its definition and historical context highlight its significance in scientific research, providing the groundwork necessary as we further explore the complexities of spectroscopic instrumentation.
Fundamental Principles of Spectroscopy
Spectroscopy serves as the bedrock of many scientific disciplines, providing methods to analyze and interpret the interaction of light with matter. Understanding these fundamental principles is crucial in grasping how various spectroscopic techniques operate. These principles illuminate the mechanisms through which samples absorb, emit, or scatter light, leading to valuable insights in chemical, biological, and physical studies. By delving into these concepts, we can appreciate the advanced instrumentation that has evolved to harness these interactions effectively.
Interaction of Light and Matter
At the heart of spectroscopy lies the interaction of light and matter. When light encounters a substance, it can be absorbed, reflected, transmitted, or scattered. This interaction depends on the composition, structure, and electronic state of the material. Essentially, different materials will interact with light in unique ways based on their intrinsic properties.
For instance, in absorption spectroscopy, specific wavelengths of light are absorbed by the substance, causing transitions in the energy levels of electrons. This alteration can be measured to identify and quantify the analytes present in a sample. Conversely, in emission spectroscopy, the sample is excited, and as it returns to a ground state, it emits light. The emitted light can then be analyzed to gather information about the composition and structure of the material.
In summary, the interaction of light and matter not only facilitates diverse spectroscopic techniques but also underpins the analysis and application of these methods in research and industry. Thus, understanding these interactions is crucial for operating spectroscopy instrumentation effectively.
Types of Spectroscopic Signals
The signals produced during spectroscopic measurements play a pivotal role in discerning the characteristics of the analyzed material. There are several types of spectroscopic signals that can arise from the interactions between light and matter. Each type provides specific information that applies to different analytical scenarios.
Absorption Signals
Absorption signals arise when light is absorbed by a sample. The resulting spectrum displays peaks at wavelengths corresponding to electronic transitions within the material. These signals reveal valuable information such as concentration and specific molecular identity.
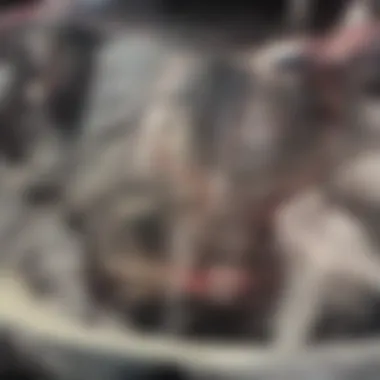
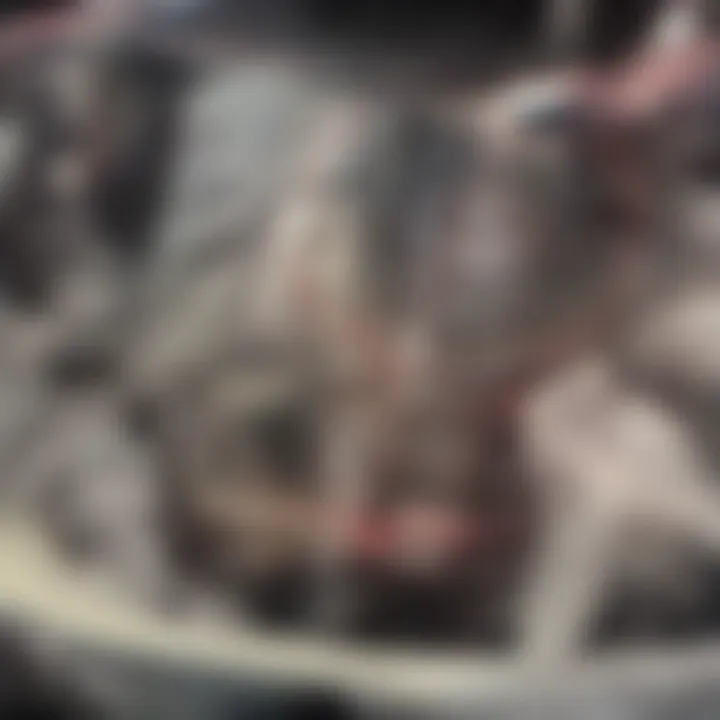
Emission Signals
Emission signals occur when a substance, after being excited by energy (such as heat or light), returns to a lower energy state, emitting light in the process. Such signals can further be subdivided into:
- Fluorescence Spectroscopy: This occurs when a sample emits light shortly after absorption. The emitted light is typically of longer wavelength than the absorbed light.
- Phosphorescence Spectroscopy: Here, the sample also emits light but with a delayed time after excitation, due to the transition through triplet states.
Raman Signals
Raman spectroscopy provides a signal based on inelastic scattering of light, known as Raman scattering. The intensity and frequency of the scattered light differ from the incident light, offering insights into molecular vibrations and bonding characteristics.
Mass Spectrometry Signals
In mass spectrometry, ionized samples are measured based on their mass-to-charge ratios. These signals allow for the identification of various compounds, aiding in complex molecular analysis.
Understanding the types of spectroscopic signals is vital for interpreting the analytical data yielded by spectroscopic techniques. Each signal type imparts critical information necessary for various fields, from pharmaceuticals to environmental science.
"Spectroscopy, through its various facets, continues to be an indispensable tool for scientific progress across disciplines."
Types of Spectroscopy
Types of spectroscopy are fundamental for understanding the interaction of light with matter. Each type offers unique insights into the physical and chemical properties of substances. This section aims to elaborate on the mechanisms, advantages, and specific applications of various spectroscopic techniques, providing a clear perspective on how these methods contribute to scientific discovery.
Absorption Spectroscopy
Absorption spectroscopy focuses on the light absorbed by a sample. When a beam of light passes through a substance, certain wavelengths are absorbed, depending on the electronic structure of the molecules present. The intensity of absorbed light is measured, allowing for the determination of the concentration and identity of the absorbing species.
This technique is beneficial for qualitative and quantitative analysis. It is commonly used in chemistry for analyzing solutions, although it can also be applied to gases and solids. The equipment required for absorption spectroscopy is generally more straightforward compared to more complex methods, making it accessible for many laboratories. Applications include the study of chemical compounds and monitoring environmental pollutants.
Emission Spectroscopy
Emission spectroscopy measures the light emitted by a sample when it returns to its ground state after excitation. This light can provide insights into the electronic structure of the molecules involved. There are two primary subtypes of emission spectroscopy: fluorescence spectroscopy and phosphorescence spectroscopy.
Fluorescence Spectroscopy
Fluorescence spectroscopy is a powerful tool for analyzing materials. It measures the short-lived emissions following excitation by a light source. The key characteristic of fluorescence is its quick response; often occurring in nanoseconds following light absorption.
Its contribution to this article lies in its usefulness for detecting low concentrations of substances. Fluorescence is popular in biological research due to its high sensitivity and the ability to label specific molecules. The unique feature is that it can be used in real-time monitoring of biological processes, making it invaluable in fields such as molecular biology and biochemistry. However, it can be affected by environmental factors such as pH and temperature, which may influence the accuracy of measurements.
Phosphorescence Spectroscopy
Phosphorescence spectroscopy analyzes light emitted over an extended period, sometimes seconds or minutes after stimulation. The key characteristic of phosphorescence is its delayed emission, which results from a change in the spin state of excited electrons, causing them to trap energy.
This method is beneficial when investigating materials that exhibit prolonged afterglow. It finds applications in solid-state physics and in studies of novel materials. The unique feature of phosphorescence is its ability to explore the energy levels of excited states over longer timescales. However, its longer emission duration may lead to challenges, like distinguishing from background signals, which could complicate analysis.
Raman Spectroscopy
Raman spectroscopy relies on inelastic scattering of monochromatic light, typically from lasers, to gain information about molecular vibrations. When light interacts with a sample, it can scatter in various ways, including a shift in energy that corresponds to the vibrational modes of the molecules.
This technique is non-destructive, allowing for analysis of samples without altering them. It is particularly effective for studying complex mixtures and obtaining fingerprints of molecular species, which is beneficial in fields like chemistry, material science, and forensic studies. One challenge is the requirement for relatively high laser intensity, which may cause heating effects in sensitive samples.
Mass Spectrometry
Mass spectrometry is critical for determining the molecular weight and structure of compounds. It works by ionizing chemical substances and measuring their mass-to-charge ratios. This method can identify compounds with high precision and is widely used in proteomics and metabolomics.
Its main advantage lies in its sensitivity, enabling detection of trace levels of substances. Mass spectrometry can analyze complex mixtures and characterize unknown compounds, making it invaluable in pharmaceuticals and environmental analysis. However, careful sample preparation and method optimization are essential to avoid contamination or degradation of samples.
Nuclear Magnetic Resonance (NMR) Spectroscopy
Nuclear magnetic resonance spectroscopy provides detailed information about the molecular structure and dynamics of a compound. By applying a strong magnetic field and radiofrequency radiation, it detects the magnetic properties of atomic nuclei. NMR is particularly useful for organic compounds and biomolecules, offering insight into molecular connectivity and conformation.
One of its key characteristics is its non-destructive nature, allowing for analysis of samples without alteration. The ability to observe molecular interactions in solution gives NMR a unique position in structural biology and organic chemistry. However, high equipment costs and complexities in data interpretation may limit its use in some laboratories.
Instrumentation Components
Spectroscopy instrumentation is a multifaceted discipline that combines various components to achieve effective analysis of light-matter interactions. Understanding the individual components that contribute to the efficacy of the spectroscopic technique is essential, as they determine the performance and accuracy of the results. The key elements of this instrumentation include light sources, sample holders, detectors, and spectroscopy software. Each plays a vital role in obtaining clear and interpretable data.
Light Sources
The selection of an appropriate light source is critical in spectroscopy. The type of light source used can influence the intensity, quality, and wavelength range of light that interacts with the sample material. Common types of light sources include X-ray tubes, lasers, quartz lamps, and LEDs.
- X-ray Tubes: Primarily used in X-ray fluorescence spectrometry (XRF) for elemental analysis.
- Lasers: Offer monochromatic light for techniques such as Raman spectroscopy and are preferred for their high coherence.
- Quartz Lamps: Frequently used in UV-Vis spectroscopy, providing a continuous light source across the UV-visible spectrum.
- LEDs: Known for their efficiency and stability, making them suitable for portable devices.
Each light source has distinct advantages and limitations. Factors such as the sample type and the specific analysis required must be considered when selecting a light source.
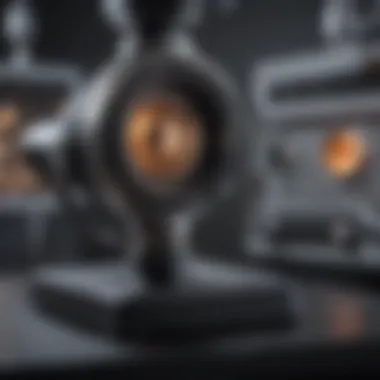
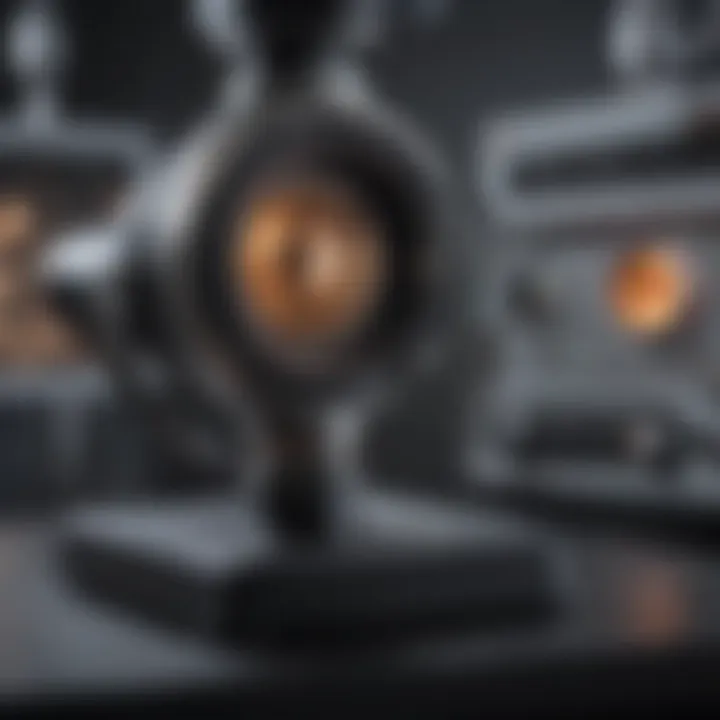
Sample Holders
Sample holders are crucial for maintaining the integrity and positioning of the sample within the optical path of the instrument. They help ensure that light can correctly interact with the sample, which is necessary for precise measurements. Common types of sample holders include:
- Cuvettes: These are used in UV-Vis spectroscopy and are usually made of glass or quartz, selected based on the light's wavelength.
- Flow Cells: Ideal for analyzing liquids in continuous flow applications.
- Solid Sample Holders: Used in FTIR spectroscopy for solid samples and can be adjusted as per the sample's size and shape.
When choosing a sample holder, considerations include the materialβs optical clarity, sample size, and the type of spectroscopic technique employed. Poor holder design can lead to scattering or absorption artifacts, thus affecting data quality.
Detectors
Detectors convert the light signal into an electrical signal, which can then be processed for analysis. The choice of detector plays a significant role in the sensitivity and resolution of the spectroscopy results. Common types of detectors include:
- Photomultiplier Tubes (PMTs): Used in emission spectroscopy. They provide high sensitivity but can be expensive.
- Charge-Coupled Devices (CCDs): These are widely used in UV-Vis and Raman spectroscopy for their ability to capture a wide range of wavelengths simultaneously.
- InGaAs Detectors: Suitable for near-infrared applications, providing high-quality results across a specific wavelength range.
- Thermocouples: Often used in molecular spectroscopy, measuring the temperature variations that correlate with sample interactions.
The choice of detector should align with the technique being utilized, ensuring optimal performance under varying experimental conditions.
Spectroscopy Software
Spectroscopy software serves as the backbone of data analysis within spectroscopic techniques. It processes the raw data collected from detectors to produce interpretable results. Key features of spectroscopy software often include:
- Data Acquisition: Efficiently captures data from various detectors and instruments in real-time.
- Data Processing: Corrects for baseline noise, performs spectral analyses, and generates necessary calculations.
- Visualization Tools: Enables users to graphically present results, making it easier to interpret complex data sets.
- Automated Tasks: Many modern software solutions offer automated calibration and validation processes, improving reproducibility.
Investing in robust spectroscopy software can significantly improve workflow efficiency and accuracy while allowing for better integration with other laboratory systems.
In summary, understanding the components of spectroscopy instrumentation is key for conducting effective experiments and obtaining reliable results.
Recent Advancements in Spectroscopy Instrumentation
Spectroscopy instrumentation has evolved significantly over the past decade. These advancements impact various fields, from fundamental research to practical applications in industry. Understanding the latest developments in spectroscopy is vital for researchers and professionals alike. This section will explore two major trends: the miniaturization of instruments and advancements in sensitivity and resolution. Both elements play crucial roles in enhancing the effectiveness of spectroscopic techniques.
Miniaturization of Instruments
The miniaturization of spectroscopy instruments is one of the most impactful trends in recent years. Smaller devices enable on-site analysis, which is invaluable in many fields, including environmental monitoring and field studies. Many researchers now have the capability to perform sophisticated analyses outside traditional laboratory settings.
Benefits of Miniaturization
- Portability: Compact designs such as handheld spectrometers allow for easy transportation and use in various locations.
- Cost-Effectiveness: Smaller instruments often require less material and resources to manufacture, which leads to lower costs for end users.
- Accessibility: With miniaturized devices, more institutions, especially in developing areas, can equip themselves with advanced analytical tools.
The miniaturization trend also focuses on integrating microfabrication techniques. Micro-electromechanical systems (MEMS) technology allows for the creation of tiny components that can enhance performance. These developments are leading towards instruments that are not only smaller but also more efficient and accurate.
Increased Sensitivity and Resolution
Increasing sensitivity and resolution of spectroscopy instruments is essential for accurate detection and analysis. As technology improves, researchers can identify compounds in lower concentrations than ever before. Enhanced sensitivity allows scientists to observe faint signals that might go unnoticed in traditional setups.
Key Aspects of Sensitivity and Resolution Improvements
- Advanced Detectors: Modern detectors like avalanche photodiodes and superconducting nanowires are offering unprecedented sensitivity levels. They can detect single photons, which is crucial for studies needing extreme precision.
- Improved Light Sources: The development of various types of lasers has contributed to higher resolution. Lasers can provide coherent light that improves the quality of spectra.
- Data Processing Technologies: Enhanced algorithms and software for signal processing have made it possible to extract reliable information from noisy data. Algorithms can separate meaningful signals from background interference, thus improving analysis outcomes.
Applications of Spectroscopy in Research
Spectroscopy is a crucial tool in various research fields, enabling scientists to analyze materials, understand reactions, and uncover new information about the universe. The significance of spectroscopy lies in its versatile applications, each serving to broaden our understanding of complex processes and structures. By studying the interaction between light and matter, researchers glean insights that inform both theoretical and practical aspects of science. This section explores the diverse applications of spectroscopy in several key domains.
Chemical Analysis
Chemical analysis through spectroscopy allows for the detailed examination of the elemental composition and structure of substances. Techniques like Absorption Spectroscopy, allow scientists to determine the concentration of compounds in a solution by measuring how much light is absorbed at specific wavelengths. This is notably useful in pharmaceutical research, where quantifying active ingredients is essential for quality control. Additionally, Mass Spectrometry plays a pivotal role in identifying chemical compounds based on their mass-to-charge ratios, providing both qualitative and quantitative data.
Key points in chemical analysis using spectroscopy include:
- Non-destructive testing methods
- High sensitivity and accuracy
- Rapid analysis capabilities
These benefits advance essential areas, such as drug development and environmental monitoring, ensuring that chemicals are analyzed accurately and efficiently.
Biological Studies
In biological research, spectroscopy offers insights into molecular interactions and cellular processes. Techniques like Fluorescence Spectroscopy are particularly powerful in studying biomolecules. This method uses fluorescence to detect and quantify specific proteins or nucleic acids, facilitating the monitoring of biological activities. For example, this technique is instrumental in studying enzyme kinetics and protein interactions, which ultimately aids in drug discovery efforts.
- Applications of spectroscopy in biological studies:
- Tracking biomolecular interactions
- Assessing drug efficacy
- Understanding metabolic pathways
Spectroscopy also allows for real-time analysis of living cells, providing valuable data without the need for invasive procedures.
Environmental Monitoring
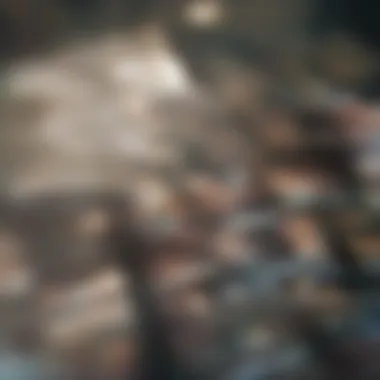
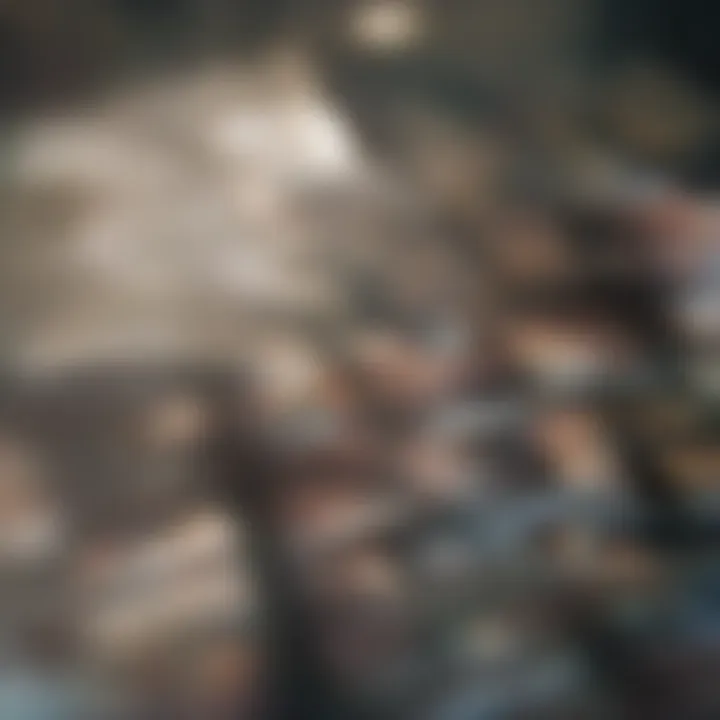
Spectroscopy plays a vital role in monitoring environmental conditions and assessing pollution levels. By employing Raman Spectroscopy or Infrared Spectroscopy, scientists can identify and quantify environmental contaminants in air, water, and soil samples. This is essential for assessing the impact of pollutants and ensuring compliance with environmental regulations.
Important aspects of using spectroscopy for environmental monitoring include:
- Real-time analysis of environmental samples
- Ability to detect low concentrations of pollutants
- Contribution to environmental protection strategies
Such capabilities enhance understanding of environmental chemistry and inform policy decisions related to public health.
Materials Science
In the field of materials science, spectroscopy is indispensable for characterizing the physical and chemical properties of materials. Techniques like Nuclear Magnetic Resonance (NMR) Spectroscopy provide insights into molecular structure and dynamics. This is vital for the design of novel materials, such as polymers and nanomaterials, and assessing their properties for specific applications.
- Common uses of spectroscopy in materials science include:
- Analyzing metallic and semiconducting materials
- Investigating material degradation
- Optimizing manufacturing processes
Spectroscopy thus enables the innovation of new materials with enhanced performance, contributing to advancements in technology and engineering.
The integration of various spectroscopic techniques opens new avenues for research and applications across disciplines, leading to greater scientific progress.
Overall, the application of spectroscopy in research domains is not just beneficial; it is essential for the continued advancement of knowledge across industries. Its ability to provide precise, rapid, and detailed information makes it a cornerstone of modern scientific investigation.
Challenges and Limitations
Understanding the challenges and limitations of spectroscopy is crucial for anyone involved in this field, from students to experienced researchers. Despite its numerous advantages, spectroscopy instrumentation faces specific hurdles that can impact its effectiveness and reliability. Addressing these challenges is vital to enhance the accuracy of measurements and the validity of results.
Signal Noise and Interference
One of the most significant challenges in spectroscopy is the presence of signal noise and interference. Spectroscopic measurements rely on detecting relatively weak signals that can be easily masked by external noise. This noise can arise from various sources, such as electrical interference, vibrations, or thermal fluctuations.
To minimize the impact of noise, researchers often utilize several strategies. These include:
- Signal Averaging: Accumulating multiple readings over time can help improve the signal-to-noise ratio, allowing clearer detection of the desired signal.
- Spectral Filtering: Filtering techniques can be applied to isolate specific frequency ranges, which can reduce unwanted background noise.
- Proper Instrument Setup: Ensuring proper alignment of optical components and minimizing stray light can also contribute to clearer signals.
Despite these methods, completely eliminating noise is seldom possible. Thus, understanding how to interpret data with noise is a skill every spectroscopist must develop.
"The challenge of signal noise impacts all forms of data analysis, making it imperative to adopt advanced techniques for accurate interpretation."
Calibration and Standardization Issues
Calibration and standardization are crucial aspects that must not be overlooked in spectroscopy. Instruments require regular calibration to ensure that they provide accurate and repeatable results. Any lapse in calibration may lead to systematic errors, rendering the data unreliable.
Common challenges include:
- Drift Over Time: Instruments can experience drift due to factors like temperature changes or aging components. Regular calibration against known standards is necessary to correct these drifts.
- Variability in Standards: The choice of calibration standards can vary between labs, which may lead to discrepancies if multiple labs are involved in research.
- Lack of Standard Protocols: In some areas of spectroscopy, defined protocols for calibration may not exist, leading to inconsistencies in data interpretation.
To mitigate these issues, researchers must develop robust calibration procedures and remain vigilant about the standards they utilize. The future of spectroscopy relies on minimizing these challenges to enhance the credibility of its applications.
In summary, while spectroscopy provides invaluable insights across various scientific disciplines, understanding its challenges is essential for advancing the field.
Future Directions in Spectroscopy
The future of spectroscopy is promising and resilient, reflecting an adaptive field of science. As technologies advance, new methodologies emerge that enhance the capabilities and applications of spectroscopic instrumentation. This evolution is crucial for addressing challenges and expanding the boundaries of what can be analyzed or measured in various scientific domains.
Integration with Other Technologies
One of the most significant future directions in spectroscopy is its integration with other technologies. This synergy can lead to improved analytics and unprecedented insight into complex systems.
- Data Science and Machine Learning: The integration of spectroscopy with data science is particularly noteworthy. Analyzing large data sets from spectroscopic measurements can reveal patterns that manual interpretation might overlook. Algorithms can improve predictive models, leading to faster and more accurate results.
- Nanotechnology: Advancements in nanotechnology enhance spectroscopy's sensitivity and resolution. New materials can provide enhanced light-matter interactions, which is beneficial in fields such as biomedical applications where detection sensitivity is paramount.
- Internet of Things (IoT): The IoT can facilitate real-time monitoring through spectroscopy. Instruments can be designed to operate within a network, allowing for seamless data collection and analysis from remote locations. This is especially useful in environmental monitoring where immediate data provides timely insights.
These integrations not only contribute to the robustness of experimental setups but also pave the way for innovative applications in industries like pharmaceuticals, environmental science, and materials engineering.
Prospects for Automation
Automation stands as another vital direction for the future of spectroscopy. The trend towards automated systems can transform how spectroscopy is implemented across laboratories and industries.
- Laboratory Efficiency: Automated sampling and analysis can greatly reduce human error and variability, making results more reliable. It also allows for high-throughput analyses, which is essential in pharmaceutical research and clinical diagnostics.
- Cost-effectiveness: Reducing the need for constant human supervision lowers operational costs. In addition, with automation, equipment usage becomes optimized, thus conserving both time and resources.
- User Accessibility: Simplifying the user interface through automation makes these sophisticated instruments more accessible to non-specialists. This democratizes the technology, permitting researchers from various fields to use spectroscopic methods without in-depth prior training.
The advancements in automation appear promising, suggesting a future where spectroscopy not only excels in accuracy and efficiency but also reaches a broader audience in its applications.
"The integration of cutting-edge technologies into spectroscopy not only enhances its capabilities but also opens doors to novel applications that were previously thought impossible."
As the field progresses, embracing these directions will be essential for maximizing the potential of spectroscopy. This convergence of technology and automation highlights the path forward, illustrating a landscape ripe for discovery and innovation.
Closure
The conclusion of this article encapsulates the vital role of spectroscopy instrumentation in modern science. It serves as a summation of the insights gathered throughout the exploration of spectroscopy techniques, their components, and applications. Understanding these concepts is crucial for students, researchers, educators, and professionals as it highlights the significance of spectroscopy across diverse scientific areas.
Through this detailed examination, it becomes evident that spectroscopy is not merely a theoretical subject. Instead, it is a practical tool that underpins a vast range of applications including chemical analysis, biological studies, and environmental monitoring. The instrumentation advancements discussed earlier, such as increased sensitivity and miniaturization, open doors for innovative research methodologies.
Moreover, the challenges highlighted remind us that while spectroscopy holds great potential, it is not without limitations. Difficulties in calibration, signal noise, and the need for integration with other technologies suggest that the field is dynamic and evolving. These factors should be considered by upcoming scientists and researchers.